
Listening and learning: noninvasive ecological research with passive acoustics
“Pay attention to the whispers, so you won’t have to listen to the screams.” – Cherokee Proverb
“Leave no trace.” – Wayne Anderson, US Forest Service
It is said that wisdom comes when we listen. In our bustling world deafened by anthropogenic engines and chatter, the sounds of nature are often drowned out. We easily mistake the wild as something rather quiet, with little to say. But one may be surprised at the amount of knowledge we can learn from paying closer attention to the muted whispers of the animals around us. Sound is a curious property of physics that, with the help of mathematics and technology, gives away clues about the spatial ecology of animals: where and when they move, feed, raise families, and survive. Simply listening allows us to gather sounds while leaving no trace, and by listening, we can uncover our collective trace on the environment around us.
As the Anthropocene extinction crisis unfolds, effective monitoring of what biodiversity we have left is increasingly imperative to catch warning signs before it is too late [1]. Traditionally, researchers have used various hands-on techniques such as survey expeditions and tag-and-release. But such methods are inherently invasive– humans are stomping into the environment– which creates practical and ethical challenges. Survey expeditions, especially into remote ecosystems, can be extremely expensive to perform and remain ineffective at detecting elusive species frightened by human presence, while tag-and-release can negatively affect the animal’s welfare [2, 3, 4]. Technological solutions, such as camera traps, face limitations in sampling, like animals noticing the cameras [5]. The ideal solution is therefore a method that is inexpensive, non-invasive, and wide-sampling. This has led to the emergence of passive acoustic monitoring (PAM) as a candidate dealbreaker.
Why acoustics? How acoustics?
PAM requires at minimum one tool: a microphone. The device is deployed at a site of interest and left to record for an extended period. That microphone records the entire acoustic landscape (a “soundscape”) of its surroundings. This includes stereotyped, or species- and behavior-specific, sounds of whatever animals frequent the vicinity and background noises that such animals encounter in their day-to-day routines. The microphone is then retrieved and the soundscape is studied. The simplicity of these steps confers several immediate advantages for PAM. It’s inexpensive in many cases, inflicts minimal disturbance onto the environment between deployment and retrieval, can cover a wide geographic distance depending on the microphones used and quantity deployed, and most importantly, measures across time– a dimension where other methods struggle.
But how can we learn so much from a soundscape alone? After all, when listening to a raw soundscape of the African savanna, we can't clearly hear an endangered bird if it’s trumpeted out by a louder elephant herd. The answer lies in the physics of sound. Sound is a vibrational wave propagated from a source through a medium. At the atomic scale, it is essentially the transfer of kinetic energy from one particle to the next in a collective oscillating fashion. This means the wave can be modified in its amplitude (how wide a wave oscillates per cycle) and frequency (number of cycles per time) through physical interactions with its environment. When one sound wave meets another, they merge to form a new wave of a modified shape. The resulting soundscape that reaches the microphone represents a single wave sculpted into a unique shape by all of its sources, still encoding information about all of them.

Now, enter signal processing. Through mathematical algorithms such as the Fast Fourier Transform, the original waves of each contributor to the audio (audio being the “signal”) can be teased out. This enables us to view the soundscape as a spectrogram, which reveals the amplitude of sounds per time at each individual level of frequency. Sounds that originated at different frequencies can be isolated in full detail. The spectrogram, in essence, allows us to “see” coexisting sounds over a long period. This is significant for ecology because different animals often vocalize at different frequencies [6], enabling us to study both the endangered bird and elephants individually within our soundscape. In addition, with microphones sensitive to frequencies beyond human hearing, we can uncover ecologically significant wildlife sounds we could have never heard on our own.
The last step is classification. Studying a species based on long-term acoustic information necessitates the identification of all of its sounds captured within a soundscape. But a PAM deployed for even a few months will have thousands of hours recorded; the figure exponentially increases if multiple microphones are used. This rendered PAM unfeasible in the past due to the sheer quantity of data to manually examine. But the issue is now resolved with a new technology on the block: deep learning. By teaching a computer to identify sounds using examples, ecologists can automate the classification process into mere hours or days. Among the most promising algorithms are convolutional neural networks (CNNs). They are specialized in image recognition, which fits well with the visual nature of spectrograms. In the past, hundreds to tens of thousands of manually classified true and false samples were required to train a CNN. These efforts nevertheless paid off through models that classify at accuracies upwards of 99% (i.e. [7]). The toil of labor is lightening as the technology rapidly advances; a study by Dufourq et al. (2022) has already identified CNNs capable of identifying primate calls at up to 82% success (F1-score) with only 25 true samples [8].
These details provide the foundation to learn much more with various experimental setups. What these setups can be, and the potential PAM has through them, is best represented through case studies.
Case 1: in situ monitoring of primate behavior
Non-human primates are among the most threatened animals in the world. At least 43% of all living non-human species are critically endangered, and 32% more are rapidly heading toward that status [9]. A primary factor to this crisis is habitat loss, namely the destruction and fragmentation of the forests most primates call home. Climate change, pollution, and human-wildlife conflict also inflict alterations to habitats as damaging as wanton logging. This can lead to resource scarcity, which imposes stressors upon primate populations that fuel subtle shifts in behavioral patterns. Monitoring these warning signs is therefore crucial for primate conservation efforts.
Most primates are highly social animals. Vocalization makes up a significant portion of communication between individuals, and as a result, some species use an extensive repertoire of behavior-stereotyped sounds (i.e. [10]). These can be documented at a spatiotemporal scale with PAM through the deployment of microphones at multiple locations. By analyzing where, when, and what sounds are detected, we can infer the function of a certain location for a population and how its use changes over time.

One example is a study of wild chimpanzee (Pan troglodytes) territories by Kalan et al. (2016). The team’s focus was on tracking the spatial distribution of two types of long-distance calls that can be heard up to 1 km away– drumming on trees and pant-hoot vocalization– and whether they indicate particular chimpanzee activities. In one of their surveys, they planted 20 acoustic devices between 1-2 km apart within a grid covering two neighboring rainforest communities in Taï National Park, Côte d’Ivoire, and recorded for 7 months. To make sure that certain calls are indeed indicative of a behavior, the scientists cross-checked their data with coexisting transect surveys conducted by a separate organization. This confirmed a positive association between drumming (the communities’ primary observed call) and chimpanzee activity that was strongest at 1 km away [11].
Spatiotemporal analysis of the soundscapes revealed two different patterns of drumming by the chimpanzee communities. Both communities exhibited the call most frequently at different spots of their territory per month, indicating where each community resided and moved. But while one community’s drumming generally coalesced close to the center of their territory, most drummings by the other occurred close to the border between both fiefs. The authors suggested this could be indicative of territorial defense, though could not confirm because some of the acoustic devices deployed away from the border were lost during national unrest [11] following a recent civil war. Nevertheless, if these calls are an indicator of intercommunity conflict, long-term PAM documentation that picks up a trend towards rising border skirmishes or unusual shifts in ranging activity could warn of a decline in habitat quality for a community. This can in turn inform early conservation intervention before chimpanzee deaths spiral.
Case 2: 3D localization of goose-beaked whales
Among the stakeholders who have found use in the expansion of PAM is the US Navy, for one reason: to stop killing whales with sonar [12]. Navy sonar operates by blasting powerful sound waves in the water and reading whatever bounces back. Unfortunately, this must be done at a frequency that can be detected by cetaceans, particularly the goose-beaked whale (Ziphius cavirostris). Starting around 2006, they began washing up dead on Pacific shorelines– sometimes with their heads bleeding profusely– following the expansion of navy sonar in the region [13]. Goose-beaked whales are specialized for a deep-sea lifestyle, attaining the record for the deepest and longest dives among all marine mammals (up to 2,992 meters [14] and 3.7 hours [15]) to catch their favorite meal of squid. The leading hypothesis holds that the whales are spooked by the sound waves and flee to the surface from the depths too quickly, causing their bodies to sustain decompression trauma under the sudden pressure change [16]. To prevent this, the navy is interested in tracking their whereabouts so they can be accounted for [12].
Goose-beaked whales spend most of their time in deep water and usually appear for only a few minutes at the surface to breathe, so traditional methods of tracking such as surface surveys and tagging are impractical. But it is an ideal situation for an acoustic approach. The species is talkative when foraging. They make clicks in frequent, consistent intervals to echolocate for squid. Moreover, they change their clicks to buzzes when chasing prey [17]. This enables a design of PAM that tracks the exact location of individual whales like a GPS.

A problem that ecologists face when deploying simple PAMs is the inability to determine the location of sound within a microphone’s hearing range. Sound dissipates as it travels from its source, so the distance between source and receiver is recorded by how much the properties of the received wave changed. Since we don’t know the properties at its source, distance is impossible to retrieve with a single microphone [18]. But what if we use multiple microphones? If we add another nearby with an overlapping range, both will receive the sound with different properties due to the differences in distance and direction. By analyzing the time difference of arrival, one receives possible locations of the source on a hyperboloid (think a blunt-tipped cone). Add one or more microphones, and more hyperboloids may be uncovered that all intersect at a singular point– the source [19].
Wiggins et al. (2012) designed a four-hydrophone (underwater microphone) array arranged in a tetrahedron apparatus. To reduce interference by seafloor noise, the array was positioned 3 meters above it. This was deployed for 25 days in a goose-beaked whale habitat off Southern California. The team was able to localize the clicks and buzzes of four individual beaked whales that dove near the array for up to 40 minutes, two of them simultaneously, and create a 3D map of their movements and prey capture attempts. During one day, a pod of dolphins passed by at the surface; the array was able to localize 2 hours worth of clicks from up to 5 km away and reconstruct the pod’s surface track [20].
The team did not stop there. If one expands the arrays, then the resulting increase in coverage of hyperboloids that can intersect onto a source can dramatically increase both the range and precision of localization. This was done in a 2015 followup study. The team’s new setup included three apparatuses of the same design and two more individual hydrophones, arranged in a cross-like formation about 0.5 km apart. This also allowed the PAM to infer the direction of a click, which the previous study could not. The study successfully mapped 11 dives by multiple beaked whales within a wider 20 km2 area, with up to three individuals foraging together side-by-side, over a 2-month period [21]. As more tracks are monitored in ongoing and future studies, scientists reach closer to the amount of information needed to delineate spatial boundaries that inform the US Navy where their sonar activities will and will not harm beaked whales.
Although small-scale, these studies demonstrate the potential of advanced PAMs. They are able to document exactly in all xyz dimensions where goose-beaked whale individuals were feeding in their natural habitat over an extended period of time without a single human-animal interaction. Localization can be applied to other wildlife whose vocal habits allow similar documentation in both the marine and terrestrial realms. The latter already includes tests to track bird migrations mid-flight [22] and could expand into more ground-based animals like individual chimpanzees as the technology further develops [11]. However, there are still limitations. Because tracking is based on connecting the dots of recorded calls, when too many individuals overlap at once, scientists can’t differentiate between them. This is why Wiggins et al. (2012) were unable to track individual dolphins within the pod. Nevertheless, each click’s acoustic properties were still captured, which allowed the calculation of parameters that can estimate population size [20].
Future of PAM: trends, challenges, and environmental justice
The above case studies provide a mere snippet of what PAM can accomplish. But as the discipline of ecological acoustics rapidly develops, its potential applications run far beyond tracking the whereabouts of an animal population. The ability for PAM to discriminate between individuals through time and, through localization, space, unlocks remote study of other biological aspects of a population such as demography and timing of life histories. This allows scientists to explore large-scale questions of climate change such as how rising temperatures alters timing of a tree’s flowering (as indicated by pollinator detections) or where their habitat range is shifting, which through indirect relationships addresses implications for the ecosystem as a whole. As a soundscape captures a holistic sample of a microphone’s surroundings, scientists can also address how the ecosystem as a unit responds to environmental change through the acoustic behavior of its constituents [23]. There are also practical applications. In the anti-poaching realm, scientists have proposed live PAM arrays to protect forest elephants by directing protection patrols and localizing gunshots to catch poachers in the act [24].

But PAM still has its limitations. An ears-only approach is deaf to the mute; animals that do not produce stereotyped sounds are inherently invisible in a soundscape. This makes acoustics impractical for fields like herpetology, where most reptiles are silent [25]. Vocal animals too can become invisible in habitats where louder noises overlap in frequency. In such situations, traditional survey methods remain the best options [23].
As a still-emerging tool, there are also challenges that future developments must tackle. One ongoing handicap is data storage. Because PAM records so much audio, their storage requirements are massive: 2 terabytes (~8 smartphones with 256-gigabyte storage) is enough to sustain hydrophones with a 200 kHz sampling rate, or 200 thousand snapshots per second, for only 2 months [26]. Although data technology is exploding amidst the smartphone revolution, ecologists cannot afford the latest innovations with the current funding available. In addition, novel products tailored for societal use are not always designed with extreme environmental resilience in mind– a 12.5-million-terabytes-per-inch quantum disk [27] is worthless to a hydrophone if it’s immediately destroyed under deep-sea pressure. As a result, ecologists generally must use technology at least a decade behind industry’s state-of-the-art (K. Frasier, pers. comm.). A shift in national attitude towards the importance of biodiversity monitoring can invest carbon tax revenue or redirect fossil fuel subsidies into ecological research to allow the affording of state-of-the-art technologies and increased collective focus on innovating environmental resilience into humanity’s best electronics for more effective PAMs.
A last consideration is one of environmental justice: accessibility. Traditional ecological research is typically centralized around a university-trained scientist as the mediator between humans and nature. This creates an unequal dynamic that minimizes the role of local and indigenous peoples and communities who have lived for generations alongside an ecosystem, and thereby exclude them from having an equitable part in the scientific effort for their own lands. But the simplistic nature of PAM’s fundamentals presents a latent potential for a democratization of ecology– anyone can deploy them, and, with rising accessibility of computers and software, study the soundscape as a spectrogram. This is further enabled by the appearance of inexpensive devices like AudioMoth, a pocket-sized 250 kHz acoustic sampler that costs as little as $50 to produce and can be fitted with custom microphones [28] and storage cards [29]. In soundscape analysis, further development of interactive visualization-based interfaces for spectrograms could provide even the most remote communities to participate in data analysis and interpretation alongside experts through a “common language” in visual sound [30]. PAM therefore has the foundation to facilitate a co-creative approach to citizen science: where the community is directly involved in every aspect of a scientific project from decision-making of design to communication of results. Communities previously excluded from the traditional model are empowered to design and conduct research as they see fit, unlocking the potential for valuable local ecological knowledge to be incorporated into the wider conservation effort [30] on equitable terms.
As challenges are addressed and the technology advances, the potential in passive acoustics as a powerful ecological tool will only increase in both what it can do and who can use it. The ability to learn so much about the world around us through sound alone– from the neighborhood park to the bottom of the sea– is an exciting concept, but it is our responsibility to use such a tool for positive change: to conserve and protect the remaining wildlife and ecosystems that threaten to disappear. Finally, its fundamental simplicity– a microphone and recording device– means that even you can perform PAM right now. It’s quite simple: go outside, reach out your smartphone into the surrounding nature with the microphone on, and listen.
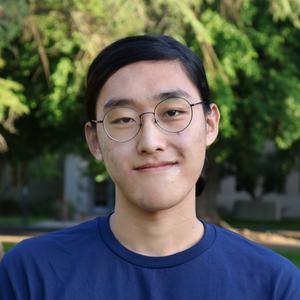
About the Author: David Kwon
David is a fourth-year Marine and Coastal Sciences major. He hopes to continue on to graduate school for an active career in research and science communication after graduating from UC Davis. Outside of The Aggie Transcript, David is often at a nearby coffee shop and enjoys drawing digital art, biking, and exploring the outdoors.
Author's Note
The aim of this feature is to provide UC Davis students a gentle but exciting introduction to the world of passive acoustics, inspired from my time as an acoustic ecology intern at the Scripps Institution of Oceanography. There, I encountered some of the wildest things we can do with a microphone, such as 3D localization, and discovered just how much potential this field has in ecology. However, I couldn’t help but notice that few institutions are tapping into its full potential. I’d like to see UC Davis, with its strong tradition in conservation and ecology, become one of them, and so hope to inspire undergrads through this feature into exploring acoustics further.
References
- Kalan AK, Mundry R, Wagner OJJ, Heinicke S, Boesch C. & Kühl HS. 2015. Towards the automated detection and occupancy estimation of primates using passive acoustic monitoring. Ecological Indicators, 54:217-226. doi:10.1016/j.ecolind.2015.02.023.
- Bodey TW, Cleasby IR, Bell F, Parr N, Schultz A, Votier SC, & Bearhop S. 2017. A phylogenetically controlled meta-analysis of biologging device effects on birds: Deleterious effects and a call for more standardized reporting of study data. Methods in Ecology and Evolution. 9(4):946-955. doi:10.1111/2041-210x.12934.
- Hawkins P. 2004. Bio-logging and animal welfare: practical refinements. Memoirs of National Institute of Polar Research, Special Issue. 58:58-68.
- Wilson R, Sala J, Gómez-Laich A, Ciancio J, & Quintana F. 2015. Pushed to the limit: food abundance determines tag-induced harm in penguins. Animal Welfare. 24(1):37-44. doi:10.7120/09627286.24.1.037.
- Garland L, Crosby A, Hedley R, Boutin S, & Bayne E. 2020. Acoustic vs. photographic monitoring of gray wolves (Canis lupus): a methodological comparison of two passive monitoring techniques. Canadian Journal of Zoology. 98(3):219-228. doi:10.1139/cjz-2019-0081.
- Martin K, Tucker MA, & Rogers TL. 2016. Does size matter? Examining the drivers of mammalian vocalizations. Evolution. 71(2):249-260.
- Zhong M, Castellote M, Dodhia R, Ferres JL, Keogh M, & Brewer A. 2020. Beluga whale acoustic signal classification using deep learning neural network models. Journal of the Acoustical Society of America. 147(3):1834-1841. doi:10.1121/10.0000921.
- Dufourq E, Batist C, Foquet R, & Durbach I. 2022. Passive acoustic monitoring of animal populations with transfer learning. Ecological Informatics. 70:101688. doi:10.1016/j.ecoinf.2022.101688.
- Estrada A, Garber PA, Rylands AB, Roos C, Fernandez-Duque E, Di Fiore A, … , & Li B. 2017. Impending extinction crisis of the world’s primates: Why primates matter. Science Advances. 3(1):e1600946. doi:10.1126/sciadv.1600946.
- Fischer J. 2021. Primate Vocal Communication and the Evolution of Speech. Current Directions in Psychological Science. 30(1):55-60. doi:10.1177/0963721420979580.
- Kalan AK, Piel AK, Mundry R, Wittig RM, Boesch C, & Kühl HS. 2016. Passive acoustic monitoring reveals group ranging and territory use: a case study of wild chimpanzees (Pan troglodytes). Frontiers in Zoology. 13:34. doi:10.1186/s12983-016-0167-8.
- Schorr GS, Falcone EA, Rone BK, Keene EL, Sweeny DA, & Coates SN. 2024. Cuvier’s beaked whale and fin whale surveys at the Southern California Offshore Anti-Submarine Warfare Range (SOAR). Seabeck, Washington: Department of the Navy. Award No’s.: N62473-19-2-0025 & N66604-22-D-F200.
- Simonis AE, Brownell RL, Thayre BJ, Trickey JS, Oleson EM, Huntington R, & Baumann-Pickering S. 2020. Co-occurrence of beaked whale strandings and naval sonar in the Mariana Islands, Western Pacific. Proceedings of the Royal Society B: Biological Sciences. 287(1921):20200070. doi:10.1098/rspb.2020.0070.
- Schorr GS, Falcone EA, Moretti DJ, & Andrews RD. 2014. First Long-Term Behavioral Records from Cuvier’s Beaked Whales (Ziphius cavirostris) Reveal Record-Breaking Dives. PLoS ONE. 9(3):e92633. doi:10.1371/journal.pone.0092633.
- Quick NJ, Cioffi WR, Shearer JM, Fahlman A, & Read AJ. 2020. Extreme diving in mammals: first estimates of behavioural aerobic dive limits in Cuvier's beaked whales. Journal of Experimental Biology. 223(18):jeb222109. doi:10.1242/jeb.222109.
- Cox TM, Ragen TJ, Read AJ, Vos E, Baird RW, Balcomb K, … , Benner L. 2005. Understanding the impacts of anthropogenic sound on beaked whales. Journal of Cetacean Research and Management. 7(3):177-187. doi:10.47536/jcrm.v7i3.
- Johnson M, Madsen PT, Zimmer WMX, de Soto NA, & Tyack PL. 2004. Beaked whales echolocate on prey. Proceedings of the Royal Society B. 271(S6):S383-S386. doi:10.1098/rsbl.2004.0208.
- Saxena A & Ng AY. 2009. Learning Sound Location from a Single Microphone. In: 2009 IEEE International Conference on Robotics and Automation. Kobe, Japan: Institute of Electrical and Electronics Engineers. 1737-1742. doi:10.1109/ROBOT.2009.5152861.
- Rhinehart TA, Chronister LM, Devlin T, & Kitzes J. 2020. Acoustic localization of terrestrial wildlife: Current practices and future opportunities. Ecology and Evolution. 10(13):6794-6818. doi:10.1002/ece3.6216.
- Wiggins SM, McDonald MA, & Hildebrand JA. 2012. Beaked whale and dolphin tracking using a multichannel autonomous acoustic recorder. Journal of the Acoustical Society of America. 131:156-163. doi:10.1121/1.3662076.
- Gassmann M, Wiggins SM, & Hildebrand JA. 2015. Three-dimensional tracking of Cuvier's beaked whales' echolocation sounds using nested hydrophone arrays. Journal of the Acoustical Society of America. 138:2483-2494. doi:10.1121/1.4927417.
- Stepanian PM, Horton KG, Hille DC, Wainwright CE, Chilson PB, & Kelly JF. 2016. Extending bioacoustic monitoring of birds aloft through flight call localization with a three-dimensional microphone array. Ecology and Evolution. 6(19):7039-7046. doi:10.1002/ece3.2447
- Ross SRP-J, O’Connell DP, Deichmann JL, Desjonquères C, Gasc A, Phillips JN, … , & Burivalova Z. 2023. Passive acoustic monitoring provides a fresh perspective on fundamental ecological questions. Functional Ecology. 37(4):959-975. doi:10.1111/1365-2435.14275
- Wrege PH, Rowland ED, Keen S, & Shiu Y. 2017. Acoustic monitoring for conservation in tropical forests: examples from forest elephants. Methods in Ecology and Evolution. 8(10):1292-1301. doi:10.1111/2041-210x.12730.
- Zwerts JA, Stephenson PJ, Maisels F, Rowcliffe M, Astaras C, Jansen PA, … , & van Kuijk M. 2021. Methods for wildlife monitoring in tropical forests: Comparing human observations, camera traps, and passive acoustic sensors. Conservation Science and Practice. 3(12):e568. doi:10.1111/csp2.568.
- Wiggins SM & Hildebrand JA. 2007. High-frequency Acoustic Recording Package (HARP) for broad-band, long-term marine mammal monitoring. Institute of Electrical and Electronics Engineers. In: International Symposium on Underwater Technology 2007 and International Workshop on Scientific Use of Submarine Cables & Related Technologies 2007. Tokyo, Japan: Institute of Electrical and Electronics Engineers. 551-557. doi: 10.1109/UT.2007.370760.
- Kim S-W, Takaya R, Hirano S, & Kasu M. 2021. Two-inch high-quality diamond heteroepitaxial growth on sapphire misoriented substrate by step-flow mode. Applied Physics Express. 14(11):115501. doi:10.35848/1882-0786/ac28e7.
- Browning E, Gibb R, Glover-Kapfer P, & Jones, KE. 2017. Passive acoustic monitoring in ecology and conservation. WWF Conservation Technology Series. 1(2). WWF-UK, Woking, United Kingdom.
- Open Acoustic Devices. Setup. Accessed April 16, 2024. Available from: https://www.openacousticdevices.info/setup-guide.
- Longdon J. 2023. Visualising Forest Sound: Justice-led Ecoacoustic Data Interaction. In: Extended Abstracts of the 2023 CHI Conference on Human Factors in Computing Systems (CHI EA '23). New York, (NY): Association for Computing Machinery. Article No.: 507. doi:10.1145/3544549.3577039.