
Current Limitations of Photodynamic Therapy for Oncological Applications and their Solutions
Introduction
The term phototherapy describes any medical treatment that incorporates light [1]. Photodynamic therapy (PDT) is a phototherapy involving light, a photosensitizer (PS), and reactive oxygen species (ROS) [2]. It is used to treat a variety of medical conditions such as psoriasis, Crohn’s disease, and more commonly acne [1]. Acne is commonly treated by PDT because the ROS generated by the PS destroys damaged skin cells [1]. PDT’s unique ability to destroy cells through oxidative stress allows it to be used for cancer treatment [2].
PDT is considered a relatively new, but effective treatment for cancer [2]. Its effectiveness is attributed to its minimal invasiveness and the variety of PS available [2]. PS variety makes PDT versatile by allowing it to treat the wide variety of existing cancers [2]. PDT is a common treatment for head and neck cancer because it is effective in penetrating tumors that are close to surface tissue [1, 3]. Despite its use for head and neck tumors, PDT is underapplied in clinical settings due to its various limitations [2].
PDT is completely reliant on oxygen saturation within tissues because it is unable to produce ROS without access to oxygen, which limits PDT’s efficacy. Tumor microenvironments along with the permeability and retention of PS also present challenges for PDT. Despite the restriction of PDT for cancer treatment, there is increasing research that aims to find solutions to its limitations. The purpose of this review is to analyze the limitations of PDT for oncological use and report current solutions to them.
PDT Process
PDT is a two-step process that consists of three parts: PS, ROS and light [1]. The PS is a drug that is locally administered to the tumor area and is specially designed to enter an excited state when exposed to light irradiance from lasers or lamps [2, 4, 5]. Once in an excited state, the PS transfers energy to molecular oxygen to form highly reactive oxygen species (ROS) such as superoxide radicals, hydroxyl radicals and hydrogen peroxide [2, 5].These ROS are cytotoxic and can kill tumor cells [5]. To effectively destroy tumors, ROS must be produced in close contact with them. Therefore, successful PDT treatment hinges on the PS being absorbed by the tumor cells so that ROS can be formed through the oxygen saturating the microenvironment.\
Delivery of PS
The delivery of PS to targeted tumor areas faces several challenges such as permeability and specificity. Although the structures of different PS varies, they are characterized by low permeability due to their hydrophobic properties [6]. This characteristic prevents it from being absorbed by tumor cells [6]. In addition to this, the specificity in which PS targets tumor cells is low, which results in the PS being mistakenly absorbed by neighboring healthy cells [6]. The PS can also be destroyed by the immune system before reaching its target [6]. A solution to the low permeability of PS is are carrier systems to administer PS [7, 8]. Carrier systems include forming micelles, spherical structures made of phospholipids, that encapsulate PS to deliver them to the tumor environment [6, 8]. Various studies found that the incorporation of PS within micelles showed improved cytotoxicity in both tumor cells and normal cells cultured under in vitro conditions [4, 6].
Although the carrier system increases PS permeability, it also increases the retention of the PS within the cells [4]; The retention of PS within cells is due to their low solubility and allows them to aggregate around cells [6]. Long-term PS retention, resulting from these PS aggregates, leads to long-term phototoxicity, which occurs when PS eventually trickle into skin cells and are then unintentionally activated by sunlight or bright lights [4, 6]. This side effect causes damage and even death to healthy cells. A solution to long-term phototoxicity is the addition of quaternary ammonium salt groups (aHP) [4]. The addition of aHP increases the molecular weight of the PS which then increases the regulation of its permeability into the cell membrane [4]. Upregulation of the PS permeability decreases the amount of time the PS is retained by cells and thus avoids long-term phototoxicity [4].
Oxygen Saturation
PDT is contingent on the generation of ROS by the PS. However, in order to produce ROS, oxygen must be present in the surrounding tissues. Low levels of oxygen saturation within cellular environments renders PDT ineffective [8]. Low oxygen saturation can be referred to as hypoxic conditions. Although hypoxic conditions can occur naturally, PDT exacerbates these conditions through its overconsumption of oxygen [8]. As a result, PDT can diminish its own efficacy.
A solution to stabilize the oxygen concentrations within cellular environments is loading micelles with oxygen-carriers [8]. The effectiveness of oxygen-carrier loaded micelles in relieving tumor hypoxia was evaluated in 2023 [8]. A block polymer was chosen to encapsulate oxygen carrier perfluoro-15-crown-5-ether (PFCE) because of its ability to assemble itself [8]. The ability of this block polymer, known as poly (ethylene glycol) methyl ether acrylate-block-n-butyl acrylate (PEGA-BA), to permeate human breast cancer cells was examined through monolayer cell culture [8]. The results of this experiment revealed that PEGA-BA loaded with PFCE was able to maintain steady oxygen concentrations while producing ample ROS to destroy cancer cells [8]. Micelles loaded with oxygen provide a viable solution to preventing PDT from exhausting oxygen concentrations.
Another solution to oxygen saturation is metronomic PDT (mPDT). PDT is typically used with high concentrations of light irradiance over a short period of time, which is known as conventional PDT (cPDT) [5, 9]. In contrast, mPDT utilizes low concentrations of light irradiance over a long period of time [5]. Evaluation of mPDT through monolayer cell culture of cancer cells demonstrated that it was more effective than cPDT at destroying cancer cells [5]. Caverzán et al came to this conclusion by measuring the cell viability of its cell cultures. Cell viability assessments are the common method to test the effectiveness of PDT [8]. mPDT also avoided activating hypoxia-inducible factors (HIF) [5]. HIF are activated in response to hypoxia therefore the ability of mPDT to avoid activating HIF indicates that mPDT does not exacerbate hypoxic conditions.
Tumor Microenvironments
As stated previously, the dependence on oxygen by PDT is one of its greatest limitations. This consumption of oxygen to generate ROS leaves tumor areas hypoxic and renders PDT ineffective. Despite the solution of loading micelles with oxygen carriers to avoid this issue, hypoxia is still a major concern [8]. Tumor microenvironments are generally very hypoxic even without PDT intervention [5]. This hypoxia contributes to the longevity and proliferation of tumor cells [5]. PDT exacerbates this issue not only by depleting oxygen levels, but also by activating hypoxia-inducible factors (HIF) that further promote tumor growth [5].
A solution to naturally occurring hypoxia in tumor microenvironments is the inclusion of bioreductive drugs within PS micelles [9]. While oxygen carriers replenish oxygen levels within tumor microenvironments, bioreductive drugs activate in the presence of hypoxic conditions [8, 9]. Bioreductive drugs develop cytotoxicity in response to hypoxia created naturally by tumor cells [8, 9]. Researchers utilized a cell culture of human breast cancer cells to measure the effectiveness of bioreductive drugs [9]. The results of their cell viability assessment revealed that the addition of a bioreductive drug within a micelle was effective in inhibiting tumor growth [9]. Future research should evaluate the effectiveness of loading a micelle with oxygen carriers and bioreductive drugs. The oxygen carriers would provide more oxygen for the photosensitizers to generate more ROS and the bioreductive drug would activate once all the oxygen is exhausted. It would be difficult to test this micelle in vivo. Therefore, this micelle should be evaluated through in vitro cell culture.
2D cell culture is one of the most common methods for testing the effectiveness of PDT as exemplified by Caverzán et al. and Li et al [5, 9]. However, this is not the most effective method for modeling tumor microenvironments. 2D cell culture lacks the complexity to display cell-cell and cell-extracellular matrix (ECM) interactions [10]. In addition, it is unable to display heterogeneity of different cell types that are naturally occurring within tumors [10]. These limitations of 2D cell culture make 3D cell culture the ideal in vitro model for studying tumor microenvironments [6].
Conclusion
PDT’s minimal invasiveness and versatility make PDT an ideal treatment for cancer. However, it is severely limited by its dependence on oxygen concentrations within tissues for its cytotoxic effects. The complexity of tumor microenvironments also provides an obstacle to the effectiveness of PDT. In addition to this, PS causes long-term phototoxicity. These limitations prevent PDT from being used in clinical cases of cancer that don’t involve head or neck tumors. Solutions to these limitations include loading micelles with oxygen-carriers and bioreductive drugs to maintain steady oxygen concentrations. Bioreductive drugs coupled with lower doses of PDT can decrease long-term phototoxicity. To ensure the success of these solutions, they should be tested with 3D cell culture of tumor cells to replicate the complexity of tumor microenvironments. A combination of all these solutions will make PDT a more effective method for cancer treatment.
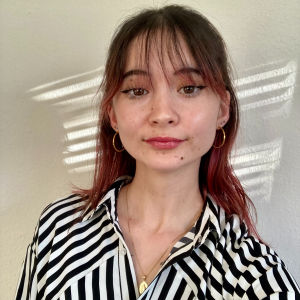
About the Author: Jazmin Orozco
I am a class of 2024 Cell Biology major. I was accepted into UC Davis as an Animal Science major. However, a graduate course on Oxidative Stress that I took during my third year along with the cell culture work I did for the Veterinary Assisted Reproduction laboratory inspired me to change my major to Cell Biology. My goal is to obtain a PhD in Cell Biology. I wrote this Literature Review as an assignment for UWP102B at UC Davis. I chose Photodynamic Therapy (PDT) because I am passionate about biomedical research for cancer treatments. PDT mixes my interest in oxidative stress with my interest in cancer treatments. I want readers to learn something new about cancer treatments. I also want them to be excited and surprised that a treatment like PDT exists for cancer.
References
Correia, J.H., Rodrigues, J.A., Pimenta, S., Dong, T., and Yang, Z. (2021). Photodynamic Therapy Review: Principles, Photosensitizers, Applications, and Future Directions. Pharmaceutics 13, 1332. https://doi.org/10.3390/pharmaceutics13091332.
Jiang, W., Liang, M., Lei, Q., Li, G., and Wu, S. (2023). The Current Status of Photodynamic Therapy in Cancer Treatment. Cancers 15, 585. https://doi.org/10.3390/cancers15030585.
Mattioli, E.J., Ulfo, L., Marconi, A., Pellicioni, V., Costantini, P.E., Marforio, T.D., Di Giosia, M., Danielli, A., Fimognari, C., Turrini, E., et al. (2022). Carrying Temoporfin with Human Serum Albumin: A New Perspective for Photodynamic Application in Head and Neck Cancer. Biomolecules 13, 68. https://doi.org/10.3390/biom13010068.
Komatsu, Y., Yoshitomi, T., Doan, V.T.H., Kurokawa, H., Fujiwara, S., Kawazoe, N., Chen, G., and Matsui, H. (2023). Locally Administered Photodynamic Therapy for Cancer Using Nano-Adhesive Photosensitizer. Pharmaceutics 15, 2076. https://doi.org/10.3390/pharmaceutics15082076.
Caverzán, M.D., Oliveda, P.M., Beaugé, L., Palacios, R.E., Chesta, C.A., and Ibarra, L.E. (2023). Metronomic Photodynamic Therapy with Conjugated Polymer Nanoparticles in Glioblastoma Tumor Microenvironment. Cells 12, 1541. https://doi.org/10.3390/cells12111541.
Gibot, L., Demazeau, M., Pimienta, V., Mingotaud, A.-F., Vicendo, P., Collin, F., Martins-Froment, N., Dejean, S., Nottelet, B., Roux, C., et al. (2020). Role of Polymer Micelles in the Delivery of Photodynamic Therapy Agent to Liposomes and Cells. Cancers 12, 384. https://doi.org/10.3390/cancers12020384.
Vermathen, M., Kämpfer, T., Nuoffer, J.-M., and Vermathen, P. (2023). Intracellular Fate of the Photosensitizer Chlorin e4 with Different Carriers and Induced Metabolic Changes Studied by 1H NMR Spectroscopy. Pharmaceutics 15, 2324. https://doi.org/10.3390/pharmaceutics15092324.
Zhang, J., Jiang, X., Luo, W., Mo, Y., Dai, C., and Zhu, L. (2023). PEGA-BA@Ce6@PFCE Micelles as Oxygen Nanoshuttles for Tumor Hypoxia Relief and Enhanced Photodynamic Therapy. Molecules 28, 6697. https://doi.org/10.3390/molecules28186697.
Li, Y., Tong, A., Niu, P., Guo, W., Jin, Y., Hu, Y., Tao, P., and Miao, W. (2022). Light-Decomposable Polymeric Micelles with Hypoxia-Enhanced Phototherapeutic Efficacy for Combating Metastatic Breast Cancer. Pharmaceutics 14, 253. https://doi.org/10.3390/pharmaceutics14020253.
- Urzì, O., Gasparro, R., Costanzo, E., De Luca, A., Giavaresi, G., Fontana, S., and Alessandro, R. (2023). Three-Dimensional Cell Cultures: The Bridge between In Vitro and In Vivo Models. IJMS 24, 12046. https://doi.org/10.3390/ijms241512046.